Atoms squished closer together than ever before, revealing seemingly impossible quantum effects
Using a clever laser technique, scientists have squished pairs of atoms closer together than ever before, revealing some truly mind-boggling quantum effects.
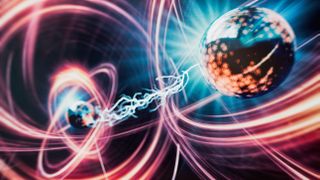
Scientists have squished two layers of ultracold magnetic atoms to within 50 nanometers of each other — 10 times closer than in previous experiments — revealing bizarre quantum effects not seen before.
The extreme proximity of these atoms will allow researchers to study quantum interactions at this length scale for the first time and could lead to important advances in the development of superconductors and quantum computers, the scientists reported in a new study published May 2 in the journal Science.
Unusual quantum behaviors begin to emerge at ultracold temperatures as the atoms are forced to occupy their lowest possible energy state. "In the nanokelvin regime, there's a type of matter called Bose Einstein condensate [in which] all the particles behave like waves," Li Du, a physicist at MIT and lead author of the study, told Live Science. "They are basically quantum mechanical objects."
Interactions between these isolated systems are particularly important for understanding quantum phenomena such as superconductivity and superradiance. But the strength of these interactions typically depends on the separation distance, which can create practical problems for researchers studying these effects; their experiments are limited by how close they can get the atoms.
"Most atoms used in cold experiments, such as the alkali metals, have to have contact in order to interact," Du said. "We're interested in dysprosium atoms which are special [in that they] can interact with each other at long range through dipole-dipole interactions [weak attractive forces between partial charges on adjacent atoms]. But although there's this long-range interaction, there are still some types of quantum phenomena that cannot be realized because this dipole interaction is so weak."
Related: Inside the 20-year quest to unravel the bizarre realm of 'quantum superchemistry'
Bringing cold atoms into close proximity while maintaining control of their quantum states is a significant challenge, and until now, experimental limitations have prevented researchers from fully testing theoretical predictions about the effects of these quantum interactions.
Sign up for the Live Science daily newsletter now
Get the world’s most fascinating discoveries delivered straight to your inbox.
"In ordinary experiments, we trap atoms with light, and that's limited by the diffraction limit — in the order of 500 nanometers," Du said. (For comparison, a human hair measures between 80,000 - 100,000 nanometers wide, according to the National Nanotechnology Initiative.)
Using a laser beam focused through a lens, researchers can create a "Gaussian focal point," which is like an energy well within the laser beam that traps particular atoms in position. This is known as an optical tweezer, but the size of the tweezer (the width of the energy well) is limited by the wavelength of the laser light. This minimum width is called the diffraction limit.
Du's team came up with a clever trick to beat this diffraction limit, using another quantum property of dysprosium atoms: their spin. Atomic spin can point either up or down — but crucially, they have slightly different energies. This means the team could use two different laser beams at slightly different frequencies and polarization angles to trap the spin-up and spin-down of dysprosium atoms separately.
"If atom A doesn't see light B and atom B doesn't see light A, they basically have independent control," he explained. "As the atoms always sit precisely at the center of the Gaussian beam, you can move [the two different trapped particles] arbitrarily close." By carefully controlling the two optical tweezers, Du's team brought the spin-up and spin-down dysprosium atoms to within 50 nanometers of each other, increasing the interaction strength by 1,000 times from 500-nanometer levels.
With this bilayer established, the team began a series of experiments to study quantum interactions at close range. They heated up one of the dysprosium layers, completely separated from the other by a vacuum gap. Incredibly, they observed heat transfer to the second layer across the empty space.
"Typically, you need contact or radiation for heat to transfer, which we don't have here," Du said. "But we still see heat transfer, and this must be due to long range dipole-dipole interactions."
Seemingly impossible heat transfer was just one of the bizarre effects the team studied. Now, they're eager to further explore the potential of quantum interactions at this scale. The group is already beginning to study how these bilayers interact with light. But Du is particularly interested in another quantum effect, called Bardeen-Cooper-Schrieffer (BCS) pairing — a quantum bound state experienced by some subatomic particles called fermions at low temperatures.
"BCS pairing between layers is very important to superconductivity," he said. "Several years ago, a theoretical paper predicted that if we have this kind of bilayer system, coupled by long range dipole-dipole interactions, you could form a BCS pair. Previously we were not able to see this experimentally, but now it could be possible with our system."
Victoria Atkinson is a freelance science journalist, specializing in chemistry and its interface with the natural and human-made worlds. Currently based in York (UK), she formerly worked as a science content developer at the University of Oxford, and later as a member of the Chemistry World editorial team. Since becoming a freelancer, Victoria has expanded her focus to explore topics from across the sciences and has also worked with Chemistry Review, Neon Squid Publishing and the Open University, amongst others. She has a DPhil in organic chemistry from the University of Oxford.